Chloroplast Protein Targeting
Robyn Goforth, Priyanka Sharma
(Lewis et al., 2010)
U of A Newswire article June 2, 2009
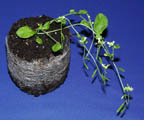
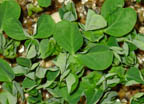
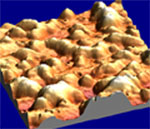
Mechanistic Studies of Protein Targeting and Integration
Introduction
Protein targeting and transport systems are fundamental to all cells. These routing systems direct proteins into or across cellular membranes from their site of synthesis to their specific sites of function within the cell. Results from work conducted over the past 20 years have uncovered an elaborate process whereby a signal recognition particle (SRP) in the cytosol is used co-translationally to target polypeptides from the cytoplasm to protein transport machinery in the ER (Keenan et al, 2001; Pool, 2003; Walter and Johnson., 1994). SRP is composed of 6 different polypeptides held around an RNA scaffold. An evolutionarily conserved 54 kDa GTPase is central to the SRP targeting mechanism. This subunit (SRP54) binds to hydrophobic signal sequences of newly synthesized polypeptides as they emerge from the ribosome, pausing translation. Affinity of SRP54 for the SRP receptor at the membrane results in targeting of the entire ribosome-mRNA-nascent polypeptide chain complex (RNC) to the ER. The SRP receptor is a heterodimer of two GTPases, SRα and SRβ. Whereas SRβ is an integral membrane protein, SRα is an evolutionarily conserved peripheral membrane protein, which binds SRP54. Ribosome binding to both SRP54 and SRα promotes their interaction at the membrane (Mandon et al., 2003), which in turn reciprocally stimulates GTP binding by both SRα and SRP54 (Powers and Walter, 2003). GTP binding by both SRP54 and SRα is required to release SRP from its targeted substrate (Rapiejko and Gilmore, 1997), but only in the presence of an appropriate and vacant translocase (Song et al., 2000). Transfer of the ribosome complex from SRP to the translocase (“Sec61”) removes translational arrest and promotes co-translational transport through the translocase. Subsequent GTP hydrolysis by SRP54 as well as SRα stimulates release of SRP from its receptor, effectively recycling SRP for additional rounds of targeting (Connolly et al., 1991).
In bacteria, where SRP is used almost exclusively to target cytoplasmic membrane proteins, an SRα homologue (“FtsY”) functions as the receptor; bacterial genomes lack a sequence homologue of SRβ. Integration of SRP targeted proteins in E. coli frequently requires a translocase (SecYEG) homologous to the Sec61 translocase in the ER. Lateral exit of membrane-spanning domains from the translocase into the lipid bilayer is mediated by an additional factor (known as “YidC” in E. coli). Importantly, YidC can also function independently of the SecYEG translocase components to promote integration of a subset of SRP-targeted membrane proteins (Froderberg et al., 2003).
Studies of protein targeting in chloroplasts lend support for a generalized model of SRP-mediated protein targeting/translocation that is evolutionarily conserved and also offers important advantages for mechanistic studies of the targeting/translocation mechanism. Our work with a post-translational chloroplast SRP (designated cpSRP) indicates that cpSRP, like other SRPs, targets substrates by interacting with a receptor and translocase at the membrane (Moore et al., 2003). The evolutionarily conserved components of the SRP targeting/translocation mechanism are shown below. Note that all SRP targeting mechanisms rely on at least two GTPases, SRP54 and SRα (Ffh and FtsY in E. coli or cpSRP54 and cpFtsY in chloroplasts).
Biochemical and genetic data demonstrate that cpSRP and its receptor (cpFtsY) are used along with GTP to target light harvesting chlorophyll binding proteins (the LHCs) to the thylakoid membrane. Similar to the situation in E. coli, the receptor partitions between the soluble and membrane phases. Biochemical and genetic studies have also demonstrated that insertion of LHCs into thylakoids requires a translocase composed minimally of Albino3 (“ALB3”), a homologue of YidC in E. coli. Nuclear encoded LHCs synthesized in the cytosol as full-length precursors are imported into the stroma where they bind cpSRP to form a soluble targeting complex termed ‘transit complex’ (Eichacker and Henry, 2001). LHCs are hydrophobic integral thylakoid proteins, and formation of a cpSRP-LHC transit complex prevents LHC aggregation in the stroma and is thought to place LHC polypeptides in an integration competent conformation (Yuan et al., 1993). CpSRP is a heterodimer composed of a conserved 54 kDa GTPase (“cpSRP54”), and a unique 43 kDa subunit “cpSRP43” (Li et al., 1995 and Groves et al., 2001).
Protein-Protein Interactions Within the Chloroplast SRP
Chloroplast SRP (cpSRP) differs from cytosolic SRPs in that it binds full-length targeting substrates, lacks an RNA moiety, and is a heterodimer composed of a conserved 54 kDa subunit, cpSRP54, and a unique 43 kDa subunit, cpSRP43. In order to efficiently target substrate to the thylakoid membrane (see overview in Mechanistic Studies of Protein Targeting and Integration -below), numerous protein-protein interactions are required. Much of our current work has focused on those interactions mediated by cpSRP43.
Model for cpSRP/cpFtsY targeting to the ALB3 translocase.
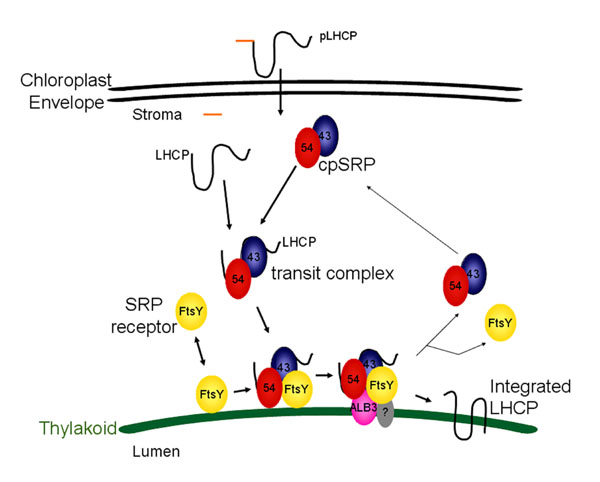
Chloroplast FtsY (yellow; FtsY), located both in the soluble phase and at the thylakoid membrane (green arc), has affinity for the ALB3 translocase. Chloroplast SRP, composed of cpSRP54 (red; 54) and cpSRP43 (blue; 43), arrives at the membrane loaded with substrate (black line, LHCP) in a guanine nucleotide-free form. CpFtsY, either bound to Albino3 (pink; ALB3) or only associated with the membrane, interacts with cpSRP to promote GTP binding by both cpSRP54 and cpFtsY. In the absence of accessible ALB3, the complex containing cpSRP, cpFtsY, and LHCP remains associated with the membrane until ALB3, and likely an additional unknown component (grey; ?), is available. There, the substrate is released to ALB3 for integration, and GTP hydrolysis liberates the cpSRP and cpFtsY for a successive round of targeting.
CpSRP43 is predicted to contain four repeated ankyrin-binding (Ank) domains and three chromatin-binding (CD) domains (Goforth et al., J Biol Chem., 2004). CDs are a family of highly conserved ~44 amino acid motifs. CD-containing proteins have been found in diverse eukaryotic organisms ranging from S. pombe to humans where CDs mediate protein-protein interactions (Eissenberg and Elgin, Curr Opin Genet Dev, 2000). Ank repeats are typically 33 residues in length where the structural repeat unit contains two antiparallel helices and a beta-hairpin. Ank domains mediate protein-protein interactions in very diverse families of proteins (Mosavi et al., PNAS., 2002).
Current interaction studies with cpSRP43 have shown the interaction of CD2 with cpSRP54 and the ankyrin region with LHCP (Goforth et al., J Biol Chem., 2004 and Jonas-Straube et al., J Biol Chem., 2001). Our present work focuses on following up on other potential interactions such as between cpSRP43 and cpFtsY. Of particular interest are those interactions involved in the regulation of GTP hydrolysis by cpSRP54/cpFtsY.
CpSRP43 chromo domains function at distinct steps during LHCP integration.
CpSRP43 is predicted to contain four repeated ankyrin-binding (Ank) domains and three chromatin-binding (CD) domains. Domains required to form a cpSRP-LHCP transit complex, required to support LHCP integration into isolated thylakoids, or that are involved in stimulation of GTP hydrolysis by cpSRP54/cpFtsY are indicated.
Characterization of the ALB3 Translocon in Chloroplasts
Introduction
Nuclear-encoded proteins destined for the chloroplast are translated in the cytosol, then imported into the plastid through proteinaceous complexes traversing the inner and outer envelope. Once inside, intrinsic properties of the protein sequence/structure seem to dictate how they will arrive at their final destination. Four transport pathways exist to localize proteins into the thylakoid membrane or lumen (Fig. 1). These have been named the cpSec pathway, which is homologous to the eukaryotic and E. coli Sec pathways; the Delta pH pathway, which led to the discovery of the Tat pathway found in prokaryotes; the cpSRP pathway, partially similar to the eukaryotic and E. coli SRP pathways; and finally the spontaneous pathway.
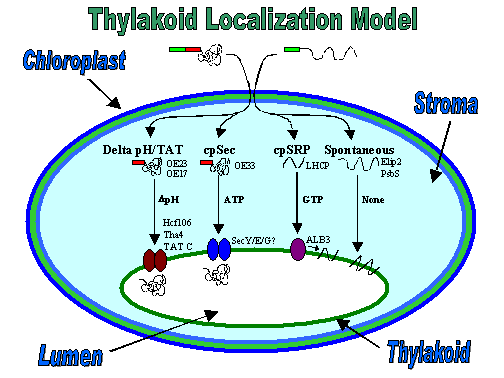
Figure 1. Precursor proteins are imported into the chloroplast, then delivered to a particular suborganellar location via one of four translocation pathways. Distinct protein and/or energetic requirements exist for each pathway as shown.
Each pathway displays distinct stromal protein and/or energetic requirements for the transport of a specific subset of proteins. The cpSec pathway requires ATP and the soluble cpSecA, a homolog of bacterial SecA, for transport of its substrates into the thylakoid lumen. Substrates include the 33 kDa subunit of the oxygen evolving complex (OE33), plastocyanin, and the chloroplast-encoded cytochrome f. No stromal proteins are required for the translocation of Delta pH pathway substrates, only a trans-thylakoid pH. These substrates include the 17 and 23 kDa subunits (OE23) of the oxygen evolving complex, and Pftf and PSII-T, the only two membrane protein substrates known for this pathway. Light harvesting chlorophyll-binding proteins, LHCPs, the only known post-translational SRP pathway substrates, utilize cpSRP (composed of cpSRP54 and cpSRP43) (1-3) and cpFtsY, an E. coli FtsY homolog, as well as GTP (4) to integrate into the thylakoid membrane (5). A subset of integral membrane proteins, including Elip2 and PsbS (6), are transported by the spontaneous insertion pathway, which is distinguished by the lack of requirement for soluble or membrane proteins as well as for nucleotides.
Components of proteinaceous pores in the membrane, termed translocons, have been identified for all but the spontaneous pathway (Table 1). The cpSec translocon is composed of at least cpSecY (7) and cpSecE (8), homologs of SecY and SecE of the bacterial SecYEG translocation pore. Hcf106 (9), Tha4 (10), and cpTatC (11) minimally comprise the Delta pH pathway pore. Finally, the cpSRP pathway uses a pore which contains ALB3 (12), a homolog of the inner membrane proteins, YidC and Oxa1p in E. coli and mitochondria, respectively.
In bacteria, YidC has been found by crosslinking and co-elution from gel filtration to associate with the SecYEG complex (13). Nascent chains of inner membrane proteins crosslink to SecY when stalled during co-translational transport. When allowed to translate further before pausing, membrane spanning regions of the same proteins crosslink to YidC. This suggests that the SecYEG translocon works in sequence with YidC. In fact, it has been hypothesized that YidC may act to facilitate the lateral transfer of transmembrane domains from the protein pore into the lipid environment (14). In contrast, proteins once thought to insert “spontaneously” into the membrane, such as M13 procoat, absolutely require YidC, but have no need for the SecYEG proteins (15).
This latter role for YidC appears to be similar to that of Oxa1 in mitochondria where there are no Sec or SRP homologs. Oxa1 is required to transport both mitochondrial and nuclear-encoded inner membrane proteins targeted from the matrix side. It has been termed the general membrane insertion machinery due to the number of proteins found to require its function for integration (16-18).
In chloroplast thylakoids, where both the cpSec pore and ALB3 function in the same membrane, no association between the two has been identified by either immunoprecipitation, crosslinking, or blue native (BN) PAGE (Moore and Henry, unpublished). Furthermore, in vitro integration of the LHCP, Lhcb1 (hereafter referred to as LHCP), was inhibited by antibodies to ALB3, but not cpSecY ((12) and described below).
Contrasting models for translocation of proteins into these three membranes are now coming into focus (Fig. 2). In E. coli, recent data describe a model where YidC is part of the SecYEG translocase during the integration of co-translationally inserted SRP-assisted membrane proteins. For those Sec-independent proteins, YidC alone seems to be necessary and sufficient (19). In contrast, ALB3 has been shown to function without the cpSec complex during SRP-dependent protein insertion. However, this was post-translational targeting to the membrane. D1, a co-translationally inserted photosystem protein is associated with cpSRP54 and targeted to SecY for translocation (20). Further experiments with this protein should show whether ALB3 is part of the Sec translocase during co-translational integration. To summarize, YidC appears to function independently or in association with the Sec translocon if the substrate requires SRP, while Oxa1p acts Sec- and SRP-independently for all substrates. ALB3 seems to have a different, but as yet not well-defined role where it works independently of the cpSec pore for the translocation of post-translationally targeted cpSRP substrates.
The identification of ALB3 translocon proteins and characterization of substrates that require ALB3 for translocation is the relevant focus of this project.
SPECIFIC GOALS
1. Identify a component of the translocon used by LHCP for post-translational integration (completed and published). Antibodies to membrane proteins (cpSecY, Hcf106, Tha4) which function as translocon components have been previously used to inhibit transport of specific pathway substrates (10). None tested were able to inhibit LHCP integration, suggesting the use of a novel pore, or lack of one. However, protease treatment of the thylakoids abolished LHCP integration, suggesting a protease-sensitive component was required. In the mitochondria, the inner membrane protein Oxa1p has proven to be a translocase component involved in integration of both mitochondrial and nuclear-encoded proteins (18). A chloroplast homolog of Oxa1p, albino3 (ALB3) was identified in Arabidopsis. ALB3 mutants are albino alluding to a role for the protein in thylakoid biogenesis (21). This information led to the idea that ALB3 could be a component of the translocon used by LHCP. To test this theory, RT-PCR was used to make cDNA clones of alb3 from both pea and Arabidopsis. Antibodies were made against a 50 amino acid region of the pea ALB3 protein (ALB3-50aa) predicted to be located on the stromal side of the thylakoid. Assays for LHCP integration were performed in vitro by combining isolated thylakoids, stromal extract (containing cpSRP and cpFtsY), GTP, and radiolabeled in vitro translated LHCP. By incubating thylakoids with anti-serum against ALB3-50aa previous to in vitro integration assays, we were able to inhibit LHCP integration by up to 84% (Fig. 3). This demonstrated a dependence on the function of ALB3 for efficient LHCP tranport into the membrane (12).
2. Examine the general role of ALB3 in thylakoid protein insertion (published). The major goal of this work will be to identify more substrate proteins for the ALB3 translocon. To date, only post-translationally targeted cpSRP-dependent light harvesting antennae proteins (LHCs) have been shown to require ALB3 (12, 25) By increasing the number of known substrates or eliminating candidate proteins, a consensus sequence or domain might be identified which targets specific proteins to the ALB3 translocon. Many potential substrates exist, including both co- and post-translationally inserted membrane proteins. By analyzing both types of substrates, the connection currently observed between cpSRP- and ALB3-dependence may be solidified or dissolved. A second benefit to finding ALB3-requiring proteins is the prospect of creating a translocation intermediate. By halting insertion, translocon components may be crosslinked to other components (ALB3), or to the translocating protein. This tool would be very valuable in the search for pore proteins.
Antibody inhibition assays, such as those used to demonstrate the involvement of ALB3 in LHCP integration, have been used to test candidate substrate proteins for their dependence on ALB3. One of the most interesting test subjects is the translocon component, cpSecE. A controlled depletion of the E. coli version, SecE, has been complemented by the thylakoid cpSecE (26). The same authors revealed that ALB3 also complements a YidC deletion. However, when bacteria deficient in both SecE and YidC were complemented with cpSecE and ALB3, the strain grew better than either complement alone suggesting that ALB3 and cpSecE work together in the membrane or one requires the other for efficient integration. Jiang et al. were also able to show that cpSecE relied on ALB3 for integration into E. coli inner membranes. We then used antibody inhibition assays to examine the reliance of cpSecE on ALB3 for integration into the thylakoid membrane. Surprisingly, we found no inhibition of cpSecE insertion when ALB3 antibodies were present. We further determined that cpSecE had no known membrane protein nor energetic requirements, thus it appears that cpSecE inserts by the “spontaneous” pathway (27).
To broaden the search for ALB3 substrates, co-translationally targeted proteins should be examined. As mentioned before, D1 protein insertion may require ALB3 in sequence with the cpSec translocon. Collaborations with other labs working with chloroplast-encoded proteins have already been discussed and should prove fruitful in this quest.
LITERATURE CITED
1. Schuenemann, D., Gupta, S., Persello-Cartieaux, F., Klimyuk, V. V., Jones, J. D. G., Nussaume, L. & Hoffman, N. E. (1998) Proc Natl Acad Sci U S A 95, 10312-6.
2. Li, X., Henry, R., Yuan, J., Cline, K. & Hoffman, N. E. (1995) Proc Natl Acad Sci U S A 92, 3789-93.
3. Klimyuk, V. I., Persello-Cartieaux, F., Havaux, M., Contard-David, P., Schuenemann, D., Meiherhoff, K., Gouet, P., Jones, J. D., Hoffman, N. E. & Nussaume, L. (1999) Plant Cell 11, 87-99.
4. Hoffman, N. E. & Franklin, A. E. (1994) Plant Physiol 105, 295-304.
5. Tu, C. J., Schuenemann, D. & Hoffman, N. E. (1999) J Biol Chem 274, 27219-24.
6. Kim, S. J., Jansson, S., Hoffman, N. E., Robinson, C. & Mant, A. (1999) J Biol Chem 274, 4715-21.
7. Laidler, V., Chaddock, A. M., Knott, T. G., Walker, D. & Robinson, C. (1995) J Biol Chem 270, 17664-7.
8. Schuenemann, D., Amin, P., Hartmann, E. & Hoffman, N. E. (1999) J Biol Chem 274, 12177-82.
9. Settles, A. M., Yonetani, A., Baron, A., Bush, D. R., Cline, K. & Martienssen, R. (1997) Science 278, 1467-70.
10. Mori, H., Summer, E. J., Ma, X. & Cline, K. (1999) J Cell Biol 146, 45-56.
11. Mori, H., Summer, E. J. & Cline, K. (2001) FEBS Lett 501, 65-68.
12. Moore, M., Harrison, M. S., Peterson, E. C. & Henry, R. (2000) J Biol Chem 275, 1529-32.
13. Scotti, P. A., Urbanus, M. L., Brunner, J., de Gier, J. W., von Heijne, G., van der Does, C., Driessen, A. J., Oudega, B. & Luirink, J. (2000) Embo J 19, 542-9.
14. Luirink, J., Samuelsson, T. & de Gier, J. (2001) FEBS Lett 501, 1-5.
15. Samuelson, J. C., Chen, M., Jiang, F., Moller, I., Wiedmann, M., Kuhn, A., Phillips, G. J. & Dalbey, R. E. (2000) Nature 406, 637-41.
16. Hell, K., Herrmann, J., Pratje, E., Neupert, W. & Stuart, R. A. (1997) FEBS Lett 418, 367-70.
17. Hell, K., Herrmann, J. M., Pratje, E., Neupert, W. & Stuart, R. A. (1998) Proc Natl Acad Sci U S A 95, 2250-5.
18. Hell, K., Neupert, W. & Stuart, R. A. (2001) Embo J 20, 1281-8.
19. Stuart, R. A. & Neupert, W. (2000) Nature 406, 575, 577.
20. Zhang L, P. V., Suorsa M, Aro EM (2001) J Biol Chem in press.
21. Sundberg, E., Slagter, J. G., Fridborg, I., Cleary, S. P., Robinson, C. & Coupland, G. (1997) Plant Cell 9, 717-30.
22. Collinson, I., Breyton, C., Duong, F., Tziatzios, C., Schubert, D., Or, E., Rapoport, T. & Kuhlbrandt, W. (2001) Embo J 20, 2462-71.
23. Neff, D. & Dencher, N. A. (1999) Biochem Biophys Res Commun 259, 569-75.
24. Schagger, H. & von Jagow, G. (1991) Anal Biochem 199, 223-31.
25. Woolhead, C. A., Thompson, S. J., Moore, M., Tissier, C., Mant, A., Rodger, A., Henry, R. & Robinson, C. (2001) J Biol Chem 276, 40841-6.
26. Froderberg, L., Rohl, T., van Wijk, K. J. & de Gier, J. W. (2001) FEBS Lett 498, 52-6.
27. Jiang, F., Yi, L., Moore, M., Chen, M., Rohl, T., Van Wijk, K. J., De Gier, J. W., Henry, R. & Dalbey, R. E. (2002) J Biol Chem 277, 19281-8.
Visualization of SRP-based Targeting at the Thylakoid Membrane
Summary
The chloroplast signal recognition particle (cpSRP) is a well studied system of protein targeting and localization to the thylakoid (1). CpSRP, unique in that it functions post-translationally (2), is used to transport a family of light-harvesting chlorophyll a/b-binding integral membrane proteins, the LHCPs. During or following import into the chloroplast, LHCP is bound by cpSRP, composed of an evolutionarily conserved 54-kDa subunit (cpSRP54) and a unique 43-kDa subunit (cpSRP43) (3,4). CpSRP transports substrates by interacting with a receptor (cpFtsY) and translocon (ALB3) at the membrane (5). Both cpSRP54 and cpFtsY are GTPases, thus GTP is required for LHCP integration (6).
While much is understood about the function and interactions of components involved in this posttranslational pathway, little is known about the three-dimensional structure and spatial arrangement of the cpSRP complex on the thylakoid membrane. In this project, we aim to use Atomic Force Microscopy (AFM) and Confocal Laser Scanning Microscopy (CLSM) in conjunction with traditional molecular biology techniques to study the cpSRP system. This will provide an enhanced picture of complicated targeting and translocation events at the membrane in a minimally modified environment. In addition, development of AFM technologies for the study of protein targeting and translocation events at the thylakoid membrane is likely to be widely applicable to other membrane and nano-biological systems.
Specific Goals
1. Image cpFtsY associated to a supported lipid bilayer membrane using Atomic Force Microscopy.
Develop a system for reconstituting Albino 3 into thylakoid-mimicking liposomes utilizing CLSM in conjunction with nano-crystal labeling.
Image ALB3 in a reconstituted, thylakoid-mimicking lipid membrane using AFM.
Image the events of the cpSRP pathway on a thylakoid-mimicking lipid membrane using AFM.
Literature Cited
1. Walter, P. & Johnson, A. E. Signal sequence recognition and protein targeting to the endoplasmic reticulum membrane. Annu Rev Cell Biol 10, 87-119 (1994).
2. Schuenemann, D. et al. A novel signal recognition particle targets light-harvesting proteins to the thylakoid membranes. Proc Natl Acad Sci U S A 95, 10312-6. (1998).
3. Groves, M. R. et al. Functional characterization of recombinant chloroplast signal recognition particle. J Biol Chem 276, 27778-86. (2001).
4. Hoffman, N. E. & Franklin, A. E. Evidence for a stromal GTP requirement for the integration of a chlorophyll a/b-binding polypeptide into thylakoid membranes. Plant Physiol 105, 295-304. (1994).
5. Moore, M., Goforth, R.L., Mori, H., & Henry, R. Functional interaction of chloroplast SRP/FtsY with the ALB3 translocase in thylakoids: substrate not required. J. Cell Bio 162,1245-1254 (2003).
6. Yuan, J. et al. ATP stimulates signal recognition particle (SRP)/FtsY-supported protein integration in chloroplasts. J Biol Chem 277, 32400-4. (2002).
CLSM images of thylakoids
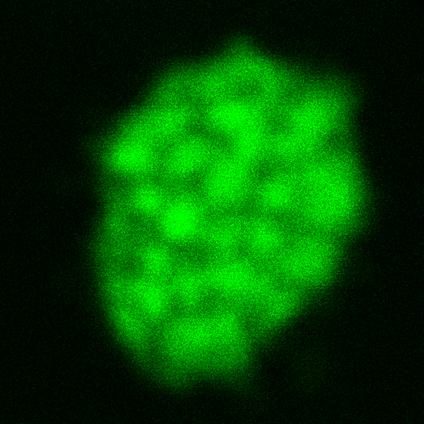
Figure 1: Autofluorescence of thylakoids imaged by CLSM – Thylakoids isolated from pea plant chloroplasts were salt-washed to remove peripherally bound proteins and then imaged using CLSM. Excitation wavelength was 488 nm. Emitted light was collected from 620-700 nm, the traditional emission range for chlorophyll. Grana are clearly seen as spots of higher intensity.
Figure 2: ALB3 tagged with Quantum Dot nano-crystals imaged by CLSM – Thylakoids isolated from pea plant chloroplasts were salt-washed to remove peripherally bound proteins and then incubated with two different ALB3 rabbit antibodies. Anti-50aa is specific to a 50 amino acid, exterior loop region of the protein. Anti-CTerm is specific to the exposed C-terminal end of the protein. After incubation with primary antibody, thylakoids were incubated with QDot 525 goat-anti-rabbit secondary antibody conjugate, which will bind to the primary antibodies and “tag” ALB3 with the QDots. Samples were excited at 488 nm. (Left) Thylakoid (green) emission was collected from 620-700 nm. QDot (red) emission was collected from 510-540 nm. (Right) After a few minutes, the thylakoids photobleach, leaving only the red fluorescence of the QDots.
AFM Images of teh thylakoid membrane
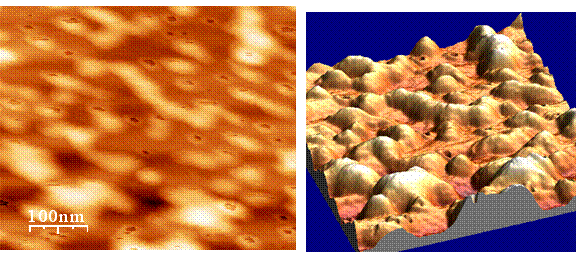
Figure 3: AFM images of thylakoids – Thylakoids isolated from pea plant chloroplasts were salt-washed to remove peripherally bound proteins and then imaged by tapping mode AFM on freshly cleaved mica in air. (Left) A height map image where color equates to surface height (lighter = higher). (Right) a three-dimensional rendering of the image on the left.
Therapeutic Anti-Methamphetamine Antibodies from Plants
FUNDING: National Institute on Drug Abuse (NIDA/NIH), $1,242,211 for 5 years (9/01 to 8/06).
PI: Dr. Ralph Henry; Co-PI: Dr. Joshua Sakon (U of A).
Dr. Mike Owens (UAMS) is the PI for the Program Project entitled
“Preclinical Testing of Antibody Therapy for (+)METH Abuse” (total $5,522,972).
The goal of this component of the program project is to develop a plant-based production system for generating medically safe and affordable pharmaceutical grade monoclonal antibodies against (+)methamphetamine ((+)METH). While antibody therapy shows tremendous promise in the treatment of (+)METH overdose and addiction, the cost to produce these antibodies using current technologies is expected to prohibit their wide-spread availability. The use of a plant-based production system will reduce the cost of antibody production by at least 10 fold. We have proposed research that will lead to expression and purification of gram quantities of anti-(+)METH IgG from plants, which are designed to be immunologically silent in therapeutic applications. Experiments will then be conducted with IgG from plants and from bioreactor cultured hybridoma cells (provided by the Core) to characterize their in vitro structural and functional equivalence. These studies will include a characterization of antibody affinity for (+)METH, antibody size, the presence or absence of glycosylation, and antibody purity. Finally, this line of investigation will culminate with the production of a high-resolution structural model of antibody (for plant and bioreactor produced) bound to (+)METH. As suitable quantities become available, IgG from plants will be used in animal models of (+)METH abuse to examine its pharmacological bioequivalence. These studies will be conducted by personnel at the Core facility utilizing behavioral and pharmacokinetic measures developed by other components of the program project. The preclinical testing of plant-produced IgG by other components in the program project should provide the critical proof needed to progress to the next stage of preclinical or clinical trials of anti-(+)METH IgG from plants.
Development of Plant-Based Production of Anti-PCP Antibody
Molecular and Biological Engineering of Plant-derived Antibodies
FUNDING: National Science Foundation (NSF), $498,920 ($997,840 with matching funds from the state of Arkansas) for 2 years beginning July 1999.
PI: Dr. Mike Owens (UAMS); Co-PIs: Dr. Ralph Henry and Dr. Brad Murphy (U of A).
U of A’s portion of the grant $247,000 from NSF (plus an additional $247,000 from state match.)
The goal of this project is to develop the infrastructure and technology to produce safe and relatively inexpensive antibody-based medicines in plants. The cost and the potential capacity of current antibody and protein production techniques are limiting factors for the use of the pharmaceutical agents in many important medical applications. However, recent breakthroughs in the processes for bioengineering of mammalian proteins in plants suggest our nation’s vast agricultural resources could be used for large-scale pharmaceutical manufacturing of protein-based medicines. Consequently, this project will be used to develop the molecular and biotechnological methods for producing pharmaceutical grade proteins in plants. To test the feasibility of the approach, plant-derived antibodies (IgG) will be generated against a prototypic drug of abuse, phencyclidine. These antibodies will then be fully characterized as pharmaceutical preparations for eventual testing in animals to determine their potential for treating the medical problems associated with drug abuse. Anti-phencyclidine murine monoclonal antibodies have been generated and extensively characterized by Mike Owens’ group at UAMS (http://www.uams.edu/pharmtox/mowens.htm) and have proven to be effective antagonists for treating acute and chromic drug abuse related medical problems in animal models of drug abuse. Production of the anti-phencyclidine antibodies in plants would set precedent for the agricultural generation of other important protein-based medicines. The agricultural development component of this research will be initially carried out in Arabidopsis, then the technology transferred to a different plant species for larger scale production. For the medical component of this research, purification techniques will be developed and the products will be extensively characterized as potential pharmaceutical preparations. This project provides and excellent prototypic system and scientific team to examine the potential for mass production of medically useful proteins in transgenic plants.
Structure-Based Antigen Binding Studies
Structure-Based Antigen Binding Studies
“UA team’s tobacco snags methamphetamine in blood” Arkansas Democrat Gazette Article August 2004
Plant-Derived Medicines Article in the University of Arkansas publication “Research Frontiers“ Fall 2000